This blog contains information regarding the building blocks of our Universe. The subjects discussed here are physics-related and I will do my best to make them as clear and as straight-forward as I can.
luni, 26 iulie 2010
Nuclear Reactor Coolant
duminică, 25 iulie 2010
Neutron Moderator
Nuclear Fuel
duminică, 11 iulie 2010
Types of Nuclear Reactors
There are several types of nuclear reactors, below I will describe some of them that are used today.
Nuclear Reactor
- Nuclear fuel
- Nuclear reactor core
- Neutron moderator
- Coolant
- Control rods
- Reactor vessel
- Boiler feedwater pump
- Steam generators
- Steam turbine
- Electrical generator
- Spent fuel pool
- Nuclear safety systems
- Containment building
- Control room
sâmbătă, 10 iulie 2010
Fission bomb
joi, 8 iulie 2010
Fission energetics
Fission mechanics
Nuclear fission can occur without neutron bombardment, as a type of radioactive decay. This type of fission (called spontaneous fission) is rare except in a few heavy isotopes. In engineered nuclear devices, essentially all nuclear fission occurs as a "nuclear reaction" — a bombardment-driven process that results from the collision of two subatomic particles. In nuclear reactions, a subatomic particle collides with an atomic nucleus and causes changes to it. Nuclear reactions are thus driven by the mechanics of bombardment, not by the relatively constant exponential decay and half-life characteristic of spontaneous radioactive processes.
A great amount of nuclear reactions are known. Nuclear fission differs importantly from other types of nuclear reactions in that it can be amplified and sometimes controlled via a nuclear chain reaction. In such a reaction, free neutrons released by each fission event can trigger yet more events, which in turn release more neutrons and cause more fissions.
The chemical element isotopes that can sustain a fission chain reaction are called nuclear fuels, and are said to be fissile. The most common nuclear fuels are 235U (the isotope of uranium with an atomic mass of 235 and of use in nuclear reactors) and 239Pu (the isotope of plutonium with an atomic mass of 239). These fuels break apart into a bimodal range of chemical elements with atomic masses centering near 95 and 135 u (fission products). Most nuclear fuels undergo spontaneous fission only very slowly, decaying instead mainly via an alpha/beta decay chain over periods of millennia to eons. In a nuclear reactor or nuclear weapon, the overwhelming majority of fission events are induced by bombardment with another particle, a neutron, which is itself produced by prior fission events.
Nuclear Fission
- Controlled chain reaction: this type of reaction is present in nuclear power facilities. By all sorts of methods, the number of neutrons that bombard the target nucleus is controlled and kept under high observation.
- Uncontrolled chain reaction: this type of reaction is present in nuclear bombs, where the number of neutrons is not controlled, therefore resulting an enormous quantity of energy released in a very small space in a very short time.
K-capture
luni, 5 iulie 2010
Nuclear force
Two or more nucleons are held together by what is called nuclear forces. These strong forces are the forces that maintain stable nuclei stable.
The nuclear force is now understood as a residual effect of an even more powerful strong force, or strong interaction, which is the attractive force that binds particles called quarks together, to form the nucleons themselves. This more powerful force is mediated by particles called gluons. Gluons hold quarks together with a force like that of electric charge, but of far greater power.
The concept of a nuclear force was first quantitatively constructed in 1934, shortly after the discovery of the neutron revealed that atomic nuclei were made of protons and neutrons, held together by an attractive force. The nuclear force at that time was conceived to be transmitted by particles called mesons, which were predicted in theory before being discovered in 1947. In the 1970’s, further understanding revealed these mesons to be combinations of quarks and gluons, transmitted between nucleons that themselves were made of quarks and gluons. This new model allowed the strong forces that held nucleons together, to be felt in neighboring nucleons, as residual strong forces.
The nuclear forces arising between nucleons are now seen to be analogous to the forces in chemistry between neutral atoms called van der Waals forces. Such forces between atoms are much weaker than the electrical forces that hold the atoms themselves together, and their range is shorter, because they arise from spontaneous separation of charges inside the atom. Similarly, even though nucleons are made of quarks and gluons that are in combinations which cancel most gluon forces, some combinations of quarks and gluons nevertheless leak away from nucleons, in the form of short-range nuclear force fields that extend from one nucleon to another close by. These nuclear forces are very weak compared to direct gluon forces inside nucleons, and they extend only over a few nuclear diameters, falling exponentially with distance. Nevertheless, they are strong enough to bind neutrons and protons over short distances, and overcome the electrical repulsion between protons in the nucleus.
Liquid drop model of the nucleus
In nuclear physics, the nucleus is sometimes compared to a liquid drop of incompressible nuclear fluid. This was first proposed by George Gamow and developed by Niels Bohr and John Archibald Wheeler. The fluid is made of nucleons (protons and neutrons), which are held together by the strong nuclear force. This is a crude model that does not explain all the properties of the nucleus, but does explain the spherical shape of most nuclei. It also helps to predict the binding energy of the nucleus.
Mathematical analysis of the theory delivers an equation which attempts to predict the binding energy of a nucleus in terms of the numbers of protons and neutrons it contains. This equation has five terms on its right hand side. These correspond to the cohesive binding of all the nucleons by the strong nuclear force, the electrostatic mutual repulsion of the protons, a surface energy term, an asymmetry term and a pairing term.
The volume energy. When an assembly of nucleons of the same size is packed together into the smallest volume, each interior nucleon has a certain number of other nucleons in contact with it. So, this nuclear energy is proportional to the volume.
The surface energy. A nucleon at the surface of a nucleus interacts with fewer other nucleons than one in the interior of the nucleus and hence its binding energy is less. This surface energy term takes that into account and is therefore negative and is proportional to the surface area.
The Coulomb energy. The electric repulsion between each pair of protons in a nucleus contributes toward decreasing its binding energy.
The asymmetry energy (Pauli energy). If it wasn't for the Coulomb energy, the most stable form of nuclear matter would have N=Z, since unequal values of N and Z imply filling higher energy levels for one type of particle, while leaving lower energy levels vacant for the other type.
The pairing energy. An energy which is a correction term that arises from the tendency of proton pairs and neutron pairs to occur. An even number of particles is more stable than an odd number.
If we define A as the number of nucleons, Z the number of protons and N the number of neutrons, the mass of an atomic nucleus is given by:
where mp and mn are the rest mass of a proton and a neutron, respectively, and EB is the binding energy of the nucleus, and c is the speed of light.
This formula states that the binding energy will take the following form:
Every energy term can be calculated and introduced into the formula along with the numbers A and Z in order to calculate the binding energy.
duminică, 4 iulie 2010
Gamma Rays
Gamma radiation is an electromagnetic radiation of a very high frequency and a very short wavelength. It is symbolized by γ. These types of rays are produced by sub-atomic interactions. These radiations have a wavelength of about 10 picometers, sometimes smaller than an atom.
These rays are a form of ionizing radiation and therefore are a health hazard because they destroy living tissue.
The measure of gamma rays' ionizing ability is called the exposure:
- The coulomb per kilogram (C/kg) is the SI unit of ionizing radiation exposure, and is the amount of radiation required to create 1 coulomb of charge of each polarity in 1 kilogram of matter.
- The gray (Gy), which has units of (J/kg), is the SI unit of absorbed dose, and is the amount of radiation required to deposit 1 joule of energy in 1 kilogram of any kind of matter.
- The sievert (Sv) is the SI unit of equivalent dose, which for gamma rays is numerically equal to the gray (Gy).
- The rem is the traditional unit of equivalent dose. For gamma rays it is equal to the rad or 0.01 J of energy deposited per kg. 1 Sv = 100 rem.
Shielding from gamma rays requires large amounts of mass. They are better absorbed by materials with high atomic numbers and high density. The higher the energy of the gamma rays, the thicker the shielding required. Materials for shielding gamma rays are typically measured by the thickness required to reduce the intensity of the gamma rays by one half.
All ionizing radiation causes similar damage at a cellular level, but because rays of alpha particles and beta particles are relatively non-penetrating, external exposure to them causes only localized damage, e.g. radiation burns to the skin. Gamma rays and neutrons are more penetrating, causing diffuse damage throughout the body (e.g. radiation sickness, increased incidence of cancer) rather than burns. External radiation exposure should also be distinguished from internal exposure, due to ingested or inhaled radioactive substances, which, depending on the substance's chemical nature, can produce both diffuse and localized internal damage.
After gamma-irradiation, and the breaking of DNA double-strands, a cell can repair the damaged genetic material to the limit of its capability. However, a study of Rothkamm and Lobrich has shown that the repairing process works well after high-dose exposure but is much slower in the case of a low-dose exposure.
Beta Decay
In nuclear physics, beta decay represents the emission of a beta particle (an electron or a positron) from the nucleus. In the case of electron emission, it is referred to as beta minus (β−), while in the case of a positron emission as beta plus (β+). The emitted beta particles have a continuous kinetic energy spectrum, with energies starting from 0 to a maximum energy (Q) limited by the parent and daughter nuclear states. The most energetic beta particles are ultrarelativistic, with speeds close to the speed of light.
- β− decay
In β− decay, the weak interaction converts a neutron (n) into a proton (p) while emitting an electron (e−) and an electron antineutrino (νe):
n → p + e− + νe
This type of beta decay usually occurs in neutron rich nuclei.
- β+ decay
In β+ decay, energy is used to convert a proton into a neutron, a positron (e+) and a neutrino (νe):
energy + p → n + e+ + νe
So, unlike β−, β+ decay cannot occur in isolation, because it requires energy, the mass of the neutron being greater than the mass of the proton. β+ decay can only happen inside nuclei when the value of the binding energy of the mother nucleus is less than that of the daughter nucleus. The difference between these energies goes into the reaction of converting a proton into a neutron, a positron and a neutrino and into the kinetic energy of these particles.
- Electron capture (K-capture)
Another type of decay is the K-capture. It consists of an electron being captured from the electron cloud by the nucleus and the emission of a neutrino (anytime the β+ decay can occur energetically, it is accompanied by a K-capture). The decay can be described as:
energy + p + e− → n + ν
This decay is called the K-capture because the closest electron is located in the K shell (the electron cloud is divided into shells, each one more further than the previous one from the nucleus; they are named K, L, M, N, P and so on) and it has the grater probability of being captured by the nucleus.
Alpha Decay
The alpha decay is a type of radioactive decay in which an atomic nucleus emits an alpha particle (an alpha particle is actually an helium nucleus - see Natural Radioactive Decay). The initial nucleus transforms into a nucleus with a mass number with 4 less particles and an atomic number with 2 less particles. An alpha decay can be described by the following nuclear equation (this is an example):
238U → 234Th + α
Alpha decay, like other cluster decays, is fundamentally a quantum tunneling process. Unlike beta decay, alpha decay is governed by the interplay between the nuclear force and the electromagnetic force.
Alpha decay typically occurs in the heaviest nuclides. In theory it can occur only in nuclei somewhat heavier than nickel (element 28), where overall binding energy per nucleon is no longer a minimum, and the nuclides are therefore unstable toward spontaneous fission-type processes. In practice, this mode of decay has only been observed in nuclides considerably heavier than nickel, with the lightest known alpha emitter being the lightest isotopes of tellurium (element 52).
Alpha particles have a typical kinetic energy of 5 MeV and a speed of 15,000 km/s. This speed corresponds to about 0.05 of c ("c" being the speed of light, about 300.000 km/s).
Most of the helium produced on Earth results from alpha decays of underground deposits of uranium and thorium. Helium is brought to the surface as a byproduct of natural gas.
vineri, 2 iulie 2010
Natural Radioactive Decay
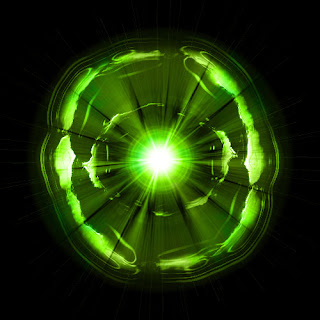
Radioactive decay is the process by which an unstable atomic nucleus loses energy by emitting ionizing particles or radiation. The emission is spontaneous in that the nucleus decays without collision with another particle. This decay, or loss of energy, results in an atom of one type, called the parent nuclide, transforming to an atom of a different type, named the daughter nuclide. For example: a carbon-14 atom (the "parent") emits radiation and transforms to a nitrogen-14 atom (the "daughter"). However given a large number of similar atoms the decay rate, on average, is predictable.
The SI unit of activity is the becquerel (Bq). One Bq is defined as one transformation (or decay) per second. A Bq is a tiny measure of activity; amounts on the order of GBq (gigabecquerel, 1 x 10.9 decays per second) or TBq (terabecquerel, 1 x 10.12 decays per second) are commonly used. Another unit of radioactivity is the curie, Ci, which was originally defined as the amount of radium emanation (radon-222) in equilibrium with of one gram of pure radium, isotope Ra-226. At present it is equal, by definition, to the activity of any radionuclide decaying with a disintegration rate of 3.7 × 10.10 Bq. The use of Ci is presently discouraged by the SI.
Radioactivity was first discovered in 1896 by the French scientist Henri Becquerel, while working on phosphorescent materials. These materials glow in the dark after exposure to light, and he thought that the glow produced in cathode ray tubes by X-rays might be connected with phosphorescence. He wrapped a photographic plate in black paper and placed various phosphorescent salts on it. All results were negative until he used uranium salts. The result with these compounds was a deep blackening of the plate. These radiations were called Becquerel Rays.
At first it seemed that the new radiation was similar to the then recently discovered X-rays. Further research by Becquerel, Marie Curie, Pierre Curie, Ernest Rutherford and others discovered that radioactivity was significantly more complicated. Different types of decay can occur, but Rutherford was the first to realize that they all occur with the same mathematical approximately exponential formula.
Types of decay:
- Alpha decay: it's seen only in heavier elements, atomic number 52, tellurium, and greater. The alpha rays carry a positive charge. From the magnitude of deflection, it was clear that alpha particles are massive. Passing alpha particles through a very thin glass window and trapping them in a discharge tube allowed researchers to study the emission spectrum of the resulting gas, and ultimately prove that alpha particles are helium nuclei.
- Beta decay: it represents the emission of one or more electrons from the nucleus. The beta rays carry a negative charge. Beta particles are much more lighter and smaller than alpha particles (these being helium nuclei). Due to their small size, the beta particles carry much more kinetic energy than the alpha particles, therefore these particles can penetrate deeper into the target material than alpha particles.
- Gamma decay: this type of decay is neutral from an electric point of view. It usually assists beta and alpha decays. Gamma rays and X-rays are both high energy electromagnetic radiation. Gamma decay (as well as beta decay) has been observed in all types of elements, not only the heavier ones.